Background science for the Turkish mutations, IV (conclusion)
In the first three posts of this series (here, here and here) we have given an overview of what the cell surface looks like to the influenza virus and set out the ideas and vocabulary virologists use to discuss the sugar molecules on the cell's surface the virus hooks on to, the viral receptor. The many possible configurations of sugars on a cell's surface serve important biological functions, like cell to cell communications and anchoring, structural uses and as receptors for important biological molecules the organism needs to function properly. These surface sugars are essential to the internal communication of the organism. But other organisms can also take advantage of them and that is how pathogens like viruses, bacteria and parasites find their way to the particular tissues and cells they need for their life cycles. Viruses use these sugar signposts as receptors to grab on to a cell in preparation for entering it and hijacking its genetic replication and protein manufacturing machinery to make new viruses.
At the risk of belaboring the point, here is yet another cartoon picture that gives the general idea (I'd credit the source if I could find it):
This picture shows the cell surface structures we have been talking about. From the first post you can see the cell membrane (the wall around the cell, also called the plasma membrane). It is a lipid bilayer, seen here as the blue and yellow archway from lower left across the picture to lower right. The blue area below it is the inside of a cell. The blue balls with yellow sticks (backed up against each other) are the phospholipid units that make up most of the cell membrane structure, but there are also some glycolipids, where instead of the blue balls you see a string of colored hexagons, which are sugar units. You see one at the left in the archway. In the first post we also talked about proteins which are anchored to and through the cell membrane. These also often have sugars attached to them, so they are called glycoproteins. There is an example second from left. The string of attached sugars is called an oligosaccharide. See the first post for details.
Items (b), (c), (d) and (e) in the picture show ways that viruses, toxins, bacteria and lymphocytes (a white cell that is part of the body's immune system) interact with glycolipids, glycoproteins and in one case, (e), an odd shaped protein that has no sugars on it but which interacts with the sugars on a glycoprotein of the lymphocyte. One of the things to notice in this stylized depiction is that the docking of the virus, the toxin or whatever, has a sort of "lock and key" aspect to it. The lock is usually a protein or glycolipid with a chain of sugars on it. The "key" is usually a protein that is shaped in just such a way that it can fit together perfectly with the sugar chain. When that kind of "perfect fit" occurs it will set in motion biological processes, often mechanisms that allow the "key" to enter into the cell or make the cell do something like manufacture a protein. Hormones work this way. They are part of the body's internal signaling system, where the signals (the hormones) travel through the blood and activate particular cells through receptors those cells have on their surfaces. Cells that don't have the receptors are unaffected. Neat system.
In the picture you can see how a virus might exploit this biological mechanism for its own purposes. If it can mimic the key of a receptor that serves another natural function (and we know only a few of these functions) it might be able to get the cell to do something useful for the virus, like set in motion processes which take the virus into the cell. But these mechanisms have to be very precise, and just like a real lock and key, if one of the bumps on the key isn't exactly right it won't work. Thus the influenza virus looks for very special sugar combinations on the cell surface. We spent most of the second post and part of the third discussing the specific signpost the virus looks for on the surface of a cell it will try to infect. These are the α-2, 3 and α-2, 6 linked Neu5AcGal two-sugar units you hear about in the flu world.
Today we look at the other side of the interaction, not the "lock side" but the "key" side, that is, the protein on the virus that fits exactly to the α-2, 3 or α-2, 6 linked Neu5AcGal units. As we noted in the third post, things now start to get really hairy. This double sugar unit is pretty specialized but it is still relatively common and is found in many species. We now know that there is a still finer structure, produced by the sugars next in line in the chain, an effect we hadn't appreciated before. Thus the next sugar might determine whether a bird virus that likes Neu5Ac α-linked to galactose through carbon #2 on Neu5Ac to carbon #3 on galactose (i.e., an α-2, 3 Neu5AcGal double sugar unit) will be more likely to infect a duck or a gull. So the basic idea is simple but its details certainly are not. What this means practically is that we still have a lot to learn about the consequences of changes in the key protein for what kind of lock it fits.
Back to the flu virus. The "key" protein, as most of you know, is the hemagglutinin (HA) protein spike that sticks through the surface of the virus. There are a lot of these spikes and they cover the surface pretty densely. Lots of "keys" to grap on to the right locks (α linked Neu5AcGal units plus whatever else might be involved we don't know about yet). The HA protein different parts and the outermost of them is called HA1 and it has a part that matches (or doesn't match) the cell surface receptor.
Now proteins are linear strings of amino acids, one linked to another, the sequence determined by the virus's genetic code. If there is a change in the genetic code, the viral protein will have a different sequence of amino acids. A change in the code is called a mutation. What is the effect of changing the sequence of the amino acids in the HA?
This turns out to be a difficult question, in general, although we know the answer in many particular cases and we are improving our abilities to predict what will happen by using computer models. One of the problems is that while the protein is one long string, like a real string it can fold into a huge number of different shapes. Proteins can be globular, ribbon shaped, elongated, threadlike, oblong or many other shapes, as the picture of the pink objects in the picture above shows. Predicting what shape a protein will take when you change the order or composition of the amino acid sequence is difficult. To show you how complicated the protein shape can be, here is a depiction of an HA protein:
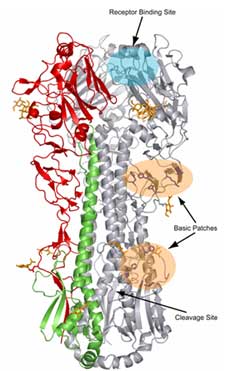
The receptor binding site is at the top, indicated with an arrow. You can see how complicated this is. And because the way the long protein chain is folded, amino acids that are very far from each other in the sequence can wind up near each other after the folding is done or wind up in a place that the shape of one amino acid might hinder binding of the Neu5AcGal unit but another one might not -- or something in between.
When we look at the sequence of amino acids in avian flu viruses, the ones that bind efficiently to α-2, 3 linkages, we find that amino acids at certain positions are important. One of these is the position numbered 223 (amino acid 223 from the start of the whole sequence; this isn't exactly correct because there are some subtleties in the numbering, but this isn't too important for our purposes). When this is the amino acid serine, the HA binds nicely to α-2, 3. But if it changes to asparagine, it switches its allegiance to α-2, 6 linkages.
Mutations are designated by the giving the amino acid before the change followed by the position and then the amino acid after the change. Hence this mutation would be designated S223N, where the S is abbreviation for serine and N the abbreviation for asparagine (you can find the standard amino acid abbreviations here). S223N is one of the mutations seen in the Turkish isolate. In other words, it is a mutation that makes it easier for the viral HA to bind to α-2, 6 Neu5AcGal, characteristic of human influenza viruses. It is not new, having been seen twice before, in a father and son in Hong Kong in 2003 and a fatal case in Vietnam last year (see Declan Butler's piece in Nature).
What Butler also revealed in Nature a few days ago, however, was that there was another mutation in the HA protein, at position 153. WHO didn't mention it because, as their spokeswoman said, "it is not clear what role this particular change plays." From this description we don't know what the mutation was, but this position is adjacent to both the receptor binding site and the site that antibodies against HA attack, i.e., it is related to whether the host's immune response can protect against the virus. Evasion of antibody response has been associated with a change at or near this site achieved by attaching a sugar there. This has been seen in viruses from Hong Kong, Indonesia, Thailand, Vietnam and Yunnan, China (Li et al. in Nature 430, 209 - 212, 2004). While WHO is correct to say the significance is not known in this case, the fact that there is a change at or near a crucial area is additional cause for concern.
Butler also reported that WHO had found a third mutation in the Turkish viruses, this one in another gene which we haven't discussed. It is associated with increased virulence in mammals, including humans, and has been found in migratory birds. Butler's news piece says this mutation might make the virus more amenable to a lower temperature, such as might be found in the nose. I don't know what data backs this up. Perhaps a reader can supply a cite. And while this mutation (E627K in the PB2 gene) has also been seen before, this is the first time all three of these mutations have been seen in the same virus.
The Nature piece concludes that there is still much to learn:
At the risk of belaboring the point, here is yet another cartoon picture that gives the general idea (I'd credit the source if I could find it):

Items (b), (c), (d) and (e) in the picture show ways that viruses, toxins, bacteria and lymphocytes (a white cell that is part of the body's immune system) interact with glycolipids, glycoproteins and in one case, (e), an odd shaped protein that has no sugars on it but which interacts with the sugars on a glycoprotein of the lymphocyte. One of the things to notice in this stylized depiction is that the docking of the virus, the toxin or whatever, has a sort of "lock and key" aspect to it. The lock is usually a protein or glycolipid with a chain of sugars on it. The "key" is usually a protein that is shaped in just such a way that it can fit together perfectly with the sugar chain. When that kind of "perfect fit" occurs it will set in motion biological processes, often mechanisms that allow the "key" to enter into the cell or make the cell do something like manufacture a protein. Hormones work this way. They are part of the body's internal signaling system, where the signals (the hormones) travel through the blood and activate particular cells through receptors those cells have on their surfaces. Cells that don't have the receptors are unaffected. Neat system.
In the picture you can see how a virus might exploit this biological mechanism for its own purposes. If it can mimic the key of a receptor that serves another natural function (and we know only a few of these functions) it might be able to get the cell to do something useful for the virus, like set in motion processes which take the virus into the cell. But these mechanisms have to be very precise, and just like a real lock and key, if one of the bumps on the key isn't exactly right it won't work. Thus the influenza virus looks for very special sugar combinations on the cell surface. We spent most of the second post and part of the third discussing the specific signpost the virus looks for on the surface of a cell it will try to infect. These are the α-2, 3 and α-2, 6 linked Neu5AcGal two-sugar units you hear about in the flu world.
Today we look at the other side of the interaction, not the "lock side" but the "key" side, that is, the protein on the virus that fits exactly to the α-2, 3 or α-2, 6 linked Neu5AcGal units. As we noted in the third post, things now start to get really hairy. This double sugar unit is pretty specialized but it is still relatively common and is found in many species. We now know that there is a still finer structure, produced by the sugars next in line in the chain, an effect we hadn't appreciated before. Thus the next sugar might determine whether a bird virus that likes Neu5Ac α-linked to galactose through carbon #2 on Neu5Ac to carbon #3 on galactose (i.e., an α-2, 3 Neu5AcGal double sugar unit) will be more likely to infect a duck or a gull. So the basic idea is simple but its details certainly are not. What this means practically is that we still have a lot to learn about the consequences of changes in the key protein for what kind of lock it fits.
Back to the flu virus. The "key" protein, as most of you know, is the hemagglutinin (HA) protein spike that sticks through the surface of the virus. There are a lot of these spikes and they cover the surface pretty densely. Lots of "keys" to grap on to the right locks (α linked Neu5AcGal units plus whatever else might be involved we don't know about yet). The HA protein different parts and the outermost of them is called HA1 and it has a part that matches (or doesn't match) the cell surface receptor.
Now proteins are linear strings of amino acids, one linked to another, the sequence determined by the virus's genetic code. If there is a change in the genetic code, the viral protein will have a different sequence of amino acids. A change in the code is called a mutation. What is the effect of changing the sequence of the amino acids in the HA?
This turns out to be a difficult question, in general, although we know the answer in many particular cases and we are improving our abilities to predict what will happen by using computer models. One of the problems is that while the protein is one long string, like a real string it can fold into a huge number of different shapes. Proteins can be globular, ribbon shaped, elongated, threadlike, oblong or many other shapes, as the picture of the pink objects in the picture above shows. Predicting what shape a protein will take when you change the order or composition of the amino acid sequence is difficult. To show you how complicated the protein shape can be, here is a depiction of an HA protein:
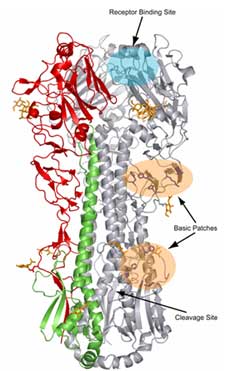
The receptor binding site is at the top, indicated with an arrow. You can see how complicated this is. And because the way the long protein chain is folded, amino acids that are very far from each other in the sequence can wind up near each other after the folding is done or wind up in a place that the shape of one amino acid might hinder binding of the Neu5AcGal unit but another one might not -- or something in between.
When we look at the sequence of amino acids in avian flu viruses, the ones that bind efficiently to α-2, 3 linkages, we find that amino acids at certain positions are important. One of these is the position numbered 223 (amino acid 223 from the start of the whole sequence; this isn't exactly correct because there are some subtleties in the numbering, but this isn't too important for our purposes). When this is the amino acid serine, the HA binds nicely to α-2, 3. But if it changes to asparagine, it switches its allegiance to α-2, 6 linkages.
Mutations are designated by the giving the amino acid before the change followed by the position and then the amino acid after the change. Hence this mutation would be designated S223N, where the S is abbreviation for serine and N the abbreviation for asparagine (you can find the standard amino acid abbreviations here). S223N is one of the mutations seen in the Turkish isolate. In other words, it is a mutation that makes it easier for the viral HA to bind to α-2, 6 Neu5AcGal, characteristic of human influenza viruses. It is not new, having been seen twice before, in a father and son in Hong Kong in 2003 and a fatal case in Vietnam last year (see Declan Butler's piece in Nature).
What Butler also revealed in Nature a few days ago, however, was that there was another mutation in the HA protein, at position 153. WHO didn't mention it because, as their spokeswoman said, "it is not clear what role this particular change plays." From this description we don't know what the mutation was, but this position is adjacent to both the receptor binding site and the site that antibodies against HA attack, i.e., it is related to whether the host's immune response can protect against the virus. Evasion of antibody response has been associated with a change at or near this site achieved by attaching a sugar there. This has been seen in viruses from Hong Kong, Indonesia, Thailand, Vietnam and Yunnan, China (Li et al. in Nature 430, 209 - 212, 2004). While WHO is correct to say the significance is not known in this case, the fact that there is a change at or near a crucial area is additional cause for concern.
Butler also reported that WHO had found a third mutation in the Turkish viruses, this one in another gene which we haven't discussed. It is associated with increased virulence in mammals, including humans, and has been found in migratory birds. Butler's news piece says this mutation might make the virus more amenable to a lower temperature, such as might be found in the nose. I don't know what data backs this up. Perhaps a reader can supply a cite. And while this mutation (E627K in the PB2 gene) has also been seen before, this is the first time all three of these mutations have been seen in the same virus.
The Nature piece concludes that there is still much to learn:
Establishing what effects these changes are having on the epidemiology of the current outbreak is a top priority for research teams working in Turkey. "We must learn more about the mild cases and be absolutely sure of whether these viruses are behaving differently from those we have seen elsewhere," says Hay. "It is early days in terms of what we know about the viruses causing these infections."While it is the beginning of an evolving story of the Turkish mutations, it concludes our odyssey through some of the basic science behind them. Some might feel it was been one long shaggy dog story. We could have gotten there faster and more easily by just summarizing the last couple of paragraphs. But then we wouldn't have had the opportunity to survey some of the science. By doing so we had the chance to set out a lot of the basic vocabulary used in the world of research on flu, which because of events, is moving directly from lab bench to bedside.
Researchers are sequencing more strains from the Turkish cases, to see whether they share the mutations and to check for further changes. Samples were expected to arrive in London on Wed 18 Jan 2006, after being held up for more than a week in Turkey because of the Eid-ul-Adha holiday period.
<< Home